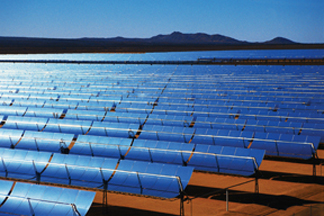
Future strategies for solar energy should follow on principles in nature, where energy is stored in chemical bonds. Some promising research into this artificial photosynthesis focuses on nanotechnology for semiconductors — essentially altering solar energy cells on the molecular scale. Image is courtesy of Corbis.
On the distant horizon is molecular nanotechnology, literally the organization
of matter at molecular scales. Imagine, for example, “smart” clothing,
in which the molecules comprising the fabric can change in response to weather.
The idea, as sketched by the late Richard Feynman at a meeting of the American
Physical Society at Caltech in 1959 and elaborated on later by many others,
is to enable the manipulation of individual atoms and molecules, using proportionally
smaller tools to build and operate even smaller tools.
Biological systems are the perfect models for molecular nanotechnology, inspiring
future nanotechnology. The capabilities of biological systems put present technology
to shame.
Although nanotechnology is often relegated to the field of materials science,
by taking a closer look at biological systems, it could one day have a profound
effect on the earth sciences. In particular, millennia-old notions of what a
“resource” is, and the collection and use of energy, are both likely
to change beyond recognition.
Fuel
laws
Current technology squanders energy because most of it is used as heat. Indeed,
we could speak of the “heat” crisis rather than the “energy”
crisis. Fuels, after all, are burned! Two-thirds of gas in an automobile’s
tank, for example, goes right out the radiator. Due to the Carnot limit, a law
that stems directly from fundamental constraints imposed by thermodynamics,
even the most efficient heat engines waste at least half the applied energy.
Because electric batteries and motors are not heat engines, they are not subject
to the Carnot limit, making them much more efficient. Conventional batteries,
however, have other engineering issues such as low energy density and slow recharge
times. Instead, fuel cells are a promising alternative.
While similar to a battery, fuel cells allow for continuous replenishment of
the reactants consumed — producing electricity from an external supply
of fuel and oxygen as opposed to relying on the limited energy storage capacity
of a battery. And contrary to popular belief, fuel cells do not necessarily
require hydrogen.
Practical fuel cells using, say, hydrocarbons or alcohols lie beyond present
technological capabilities, as converting the chemical energy of fuels directly
into electricity requires a highly controlled molecular-scale reaction. This
process requires catalysts that are both extremely specific and robust, and
hence well structured at the nanoscale. Better catalysts in general are an obvious
application of near-term nanotechnology, and will have further profound and
ramifying effects on energy efficiency.
Another near-term nanotechnology solution involves solar energy. It is often
claimed that the high energy density of conventional fuels is not reproducible
by any conceivable alternatives, at least at the scale required for modern civilization.
But the high energy density of conventional fuels is merely a brute-force solution
that is compensating for the inefficiency of burning them. Thus, it is simply
not true that solar power is incapable of powering a technological culture.
A high-tech culture is the only sort that can be run on solar power. After
all, life itself, with its extraordinary capabilities of self-organization,
synthesis and element separation, runs on solar power. That’s why it is
amusing to consider, for example, the oft-proposed use of biomass for fuel:
Burning material originally assembled, atom by atom, from diffuse sources of
both energy and materials.
Artificial photosynthesis
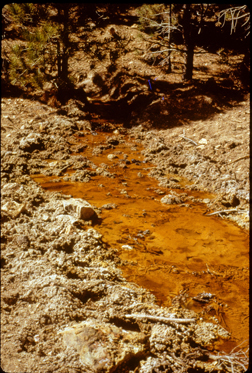
Why is
solar power usually thought to involve converting sunlight into electricity?
Biology doesn’t do it that way. Natural photosynthesis stores the energy
of sunlight in chemical bonds. That makes a lot more sense biologically, as
well as technologically.
The conventional disadvantages of solar power are that it is intermittent,
and difficult to transport and store. The last two disadvantages are true of
electricity, no matter how it is created. Using sunlight to make fuels, however,
would solve the intermittency problem: Fuel can accumulate whenever the sun
is shining and then be used later when needed.
Acidic drainage from mines is a pollution
problem that could one day be a potential resource, by using nanotechnology
to separate valuable minerals and dispose of contaminants. Photo is courtesy
of Stephen L. Gillett.
Artificial photosynthesis is now receiving much attention by industry and research
groups. The most promising approaches are based on semiconductors — materials
for controlling conductivity that make possible most of modern-day electronics,
including computer chips and lasers.
As in a conventional photovoltaic (solar) cell, semiconducting materials, such
as silicon, absorb solar radiation. That radiation knocks electrons loose to
create a flow of current, and each excited electron leaves behind a vacancy,
or “hole,” that acts like a single positive charge. Conventionally,
the electrons and holes are forced to drive an electric circuit before they
recombine. Instead of driving an external circuit, however, technical alterations
can make the hole and electron drive chemical reactions that store energy.
Most research has focused on “water splitting,” the production of
hydrogen gas from water, but alternative fuel generation is possible. Familiar
semiconductors, such as silicon, are too vulnerable to oxidation reactions to
be used in such photochemical applications. Work instead has focused on oxide
semiconductors, such as titanium dioxide, which remain stable indefinitely in
an oxygen-water environment. Reaction takes place at the wetted interface between
the water and semiconductor.
To be practical, however, such semiconductor surfaces will require near-molecular-scale
structuring. Because the electrons and holes can combine to form heat, nanostructuring
of the surface is necessary to ensure reasonable charge separation. At present,
“decorating” the surface with nanoparticles of a precious metal, such
as platinum, is the favored method of ensuring charge separation, but obviously
this increases both expense and complexity.
An ironic result of such technologies is that desert areas, with their year-round
sunlight, could become major fuel production centers. In particular, the nations
of the Middle East could continue exporting fuel indefinitely, albeit in competition
with other deserts throughout the world.
Pollutant v. Resource
A fundamental technical problem involves separating one kind of atom or molecule
from a background of others: pollutants from wastewater, metals from ores, salt
from seawater. Separation is basic to purification, pollution control and resource
extraction. Defining the process is a question of context: If we want what we
separated, it is a resource; otherwise it is a pollutant.
Traditionally, however, separation has been viewed as the source of a host
of different problems. In particular, researchers have seen resource extraction
not only as distinct from pollution control, but also as intrinsically energy-intensive.
In turn, its profligate energy usage is typically justified by vague appeals
to the laws of thermodynamics.
Yet, quantitatively, element separation is not intrinsically expensive. Do
not merely believe thermodynamic calculations: Bio-logical systems underscore
how woefully inefficient conventional separation processes are, as they perform
feats that put conventional resource extraction to shame.
Organisms do not carry out thermally driven phase separation. Instead, they
literally move individual atoms or molecules, using specialized mechanisms —
for example the binding of nutrient elements by specialized proteins. These
molecular-scale processes are vastly less costly energetically and allow separation
from considerably lower concentrations.
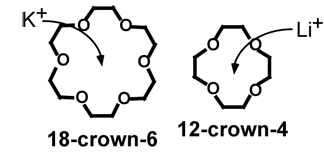
Plant
roots extract both nutrients and water at low concentrations from the ambient
soil. Vertebrate kidneys extract only certain solutes out of the blood from
a background of many other solutes. For photosynthesis, plants extract carbon
dioxide from the air, where its concentration is only about 350 parts per million,
and furthermore do so using only the diffuse and intermittent energy of sunlight.
Diatoms are particularly impressive, building shells from silica extracted at
parts-per-million levels from the ambient water.
Organic compounds called “crown
ethers,” shown here schematically, could be key players in nanotechnology
designed to extract metals. The ring, or “crown,” changes in structure
by substituting differently sized atoms, such as potassium or lithium, for oxygen
in the crown. Image is courtesy of Stephen L. Gillett.
Again, the reason why conventional resource extraction is so energy expensive
is because it largely relies on vast quantities of heat, in this case to drive
the partitioning of elements into coexisting phases. Not only do such processes
require a lot of energy, but they also are intrinsically polluting, both due
to the combustion necessary to generate the heat and because the separation
is never complete. Moreover, byproducts containing geochemically abundant elements,
such as iron in copper ores, are usually uneconomic and discarded as waste.
Thermal-based separation is also impractical for pollution control and purification.
Of course, that’s why such problems are traditionally viewed as distinct
from resource extraction. Indeed, a number of embryonic molecular separation
technologies already exist whose development has largely been driven by addressing
purification issues.
Extracting solutions
In their simplest form, molecular separation techniques require that the material
being separated be free to flow as a gas or a liquid. Selectivity of the separation
is also fundamental: Usually only one particular dissolved species is of interest,
but it is dispersed in a background of many others. Sometimes the species is
valuable (for example, palladium and lithium), whereas in other cases it is
toxic (for example, lead and cadmium).
One particular set of approaches toward selective molecular separation has
been the focus of a tremendous amount of research in recent decades. Such efforts
involve molecules with branched and ring structures that can bind tightly and
specifically only with certain solutes. For instance, a group of organic molecules
called crown ethers are highly effective extraction agents for many metal ions.
Crown ethers are strongly selective. The ring, or “crown,” changes
in structure by substituting differently sized atoms, such as nitrogen and sulfur,
for oxygen in the crown. For example, the crown ether 18-crown-6 forms a strong
complex with the potassium ion, which fits nicely into the ring, whereas the
smaller ring of 12-crown-4 strongly binds with the lithium ion, but is too small
to accommodate potassium.
One application of such a separation system is to tether the extraction agent
to a substrate to form a highly selective surface for extracting particular
solutes from solution. For example, researchers have used a substituted crown
ether tethered to a silica surface to recover palladium from scrap catalytic
converters dissolved in acid. The palladium is bound, while the other much more
abundant metals remain in solution.
The major problem with such approaches to separation is that eventually the
solute must release its captured ions to regenerate the extraction agent. Typically
this takes extreme chemical measures. In the palladium recovery system, for
example, highly concentrated acid must be used to flush out the palladium.
Such steps generate a much larger volume of wastewater that now becomes a serious
disposal problem. Separation requiring washing with fluids can be practical
for recovery of highly valuable commodities like palladium, but its applications
are obviously limited.
So-called switchable binding provides a way to solve the problem: Under one
set of circumstances, binding occurs, but changing some variable causes the
solute to unbind again. Again, biology has anticipated technology. Hemoglobin,
for example, binds strongly to oxygen in the lungs, but under different chemical
conditions elsewhere in the body, it gives up the oxygen to the tissues.
An example of switchable binding is “electrosorption,” which is based
on straightforward principles of attraction and repulsion. Charging an electrode
attracts ions with the opposite charge; reversing the charge of the electrode
desorbs the ions again. Although first proposed in the 1960s for desalination,
electrosorption remained impractical until the recent advent of nanostructured
electrodes with very high surface areas. Because the “filled up” electrodes
look like a charged capacitor, too, a great deal of the electrical energy can
be recovered when the ions are desorbed.
More selective approaches require more molecular-scale structuring. For example,
researchers have patented a process for extracting lithium ion from brine that
uses electrodes made of a form of crystalline manganese dioxide. In the process,
the electrode becomes negative, which leaves the crystal with an overall negative
charge, so positive lithium cations are drawn into tunnels in the structure
to compensate. Lithium cations can fit into these tunnels, whereas larger cations
cannot. Reversing the charge on the manganese dioxide electrode then expels
the lithium.
A similar system for extracting cesium ion is based on cesium nickel hexacyanoferrate.
Here, the crystal structure contains large cavities that can accommodate the
big cesium ion. Again, on applying a negative voltage, cesium cations are drawn
in to compensate. The system is of great interest for extracting highly radioactive
cesium-137 from nuclear waste.
An alternative potential trigger for switching binding is light. One way is
to use molecules that change their structure upon absorbing a photon. The “backbone”
of spiropyrans, a specialized class of molecules, for example, rearranges so
drastically that a solution containing the molecule actually changes color when
illuminated. Strategically arranging the extracting groups on the backbone can
make the molecule go from binding solutes in its ground state to releasing them
upon illumination.
A different approach uses the absorption of light by a semiconductor surface,
but such systems are nascent. In this case, the photogenerated electric charge
would drive molecular mechanisms at the surface. For example, a surface might
adsorb ions from a solution in the dark, but desorb those ions when illuminated.
Merely shining sunlight on a surface to desorb its solutes would obviously be
much cleaner and “greener” than flushing it with strong acid solutions!
Blurring the lines
At present, pollution control and purification are the key economic drivers
for these separation technologies. As they mature, however, they will blur the
distinction between a “pollutant” and a “resource.” Moreover,
recovered pollutants will begin to have an impact on resource extraction. After
all, copper extracted from a wastewater stream is copper that does not have
to be mined.
Ultimately, a great many aqueous solutions, of both natural and artificial
origin, will become nontraditional resources. Wastewater streams, acid-mine
drainage, seawater, concentrated natural brines such as those in oilfields or
saline lakes — sometimes viewed now as problems — all could become
potential sources of materials with the help of nanotechnology.